SRINAGAR: The Royal Swedish Academy of Sciences has decided to award the Nobel Prize in Physics 2023 to Pierre Agostini (The Ohio State University, Columbus, USA), Ferenc Krausz (Max Planck Institute of Quantum Optics, Garching and Ludwig-Maximilians-Universität München, Germany) and Anne L’Huillier (Lund University, Sweden) “for experimental methods that generate attosecond pulses of light for the study of electron dynamics in matter.”
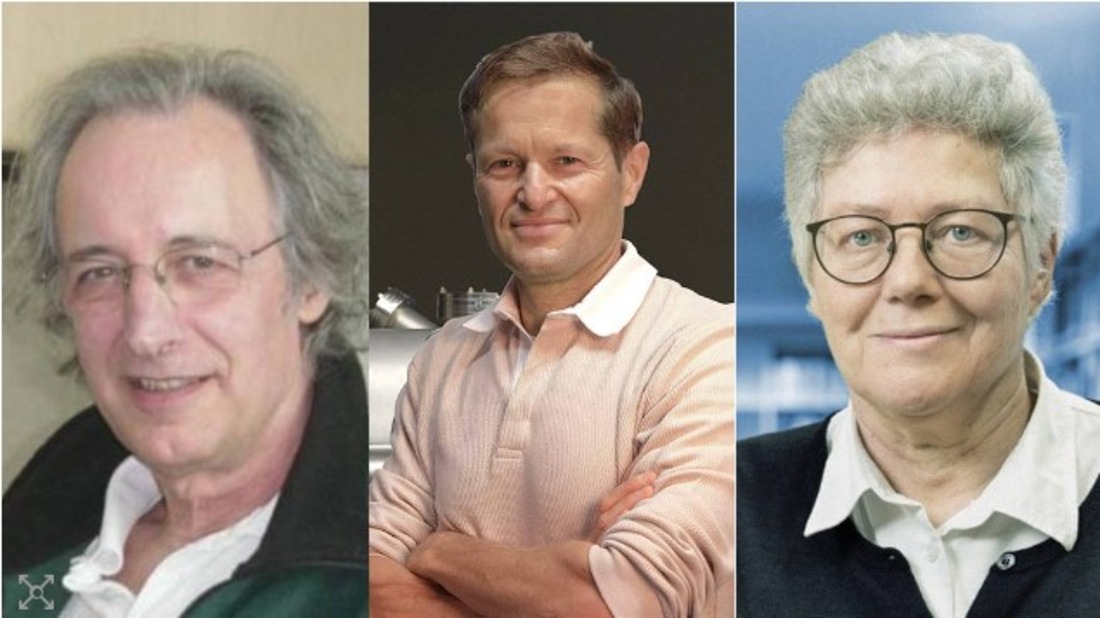
The three Nobel Laureates in Physics 2023 are being recognised for their experiments, which have given humanity new tools for exploring the world of electrons inside atoms and molecules. Pierre Agostini, Ferenc Krausz and Anne L’Huillier have demonstrated a way to create extremely short pulses of light that can be used to measure the rapid processes in which electrons move or change energy.
Fast-moving events flow into each other when perceived by humans, just like a film that consists of still images is perceived as a continual movement. If we want to investigate really brief events, we need special technology. In the world of electrons, changes occur in a few tenths of an attosecond – an attosecond is so short that there are as many in one second as there have been seconds since the birth of the universe.
The laureates’ experiments have produced pulses of light so short that they are measured in attoseconds, thus demonstrating that these pulses can be used to provide images of processes inside atoms and molecules.
In 1987, Anne L’Huillier discovered that many different overtones of light arose when she transmitted infrared laser light through a noble gas. Each overtone is a light wave with a given number of cycles for each cycle in the laser light. They are caused by the laser light interacting with atoms in the gas; it gives some electrons extra energy that is then emitted as light. Anne L’Huillier has continued to explore this phenomenon, laying the ground for subsequent breakthroughs.
In 2001, Pierre Agostini succeeded in producing and investigating a series of consecutive light pulses, in which each pulse lasted just 250 attoseconds. At the same time, Ferenc Krausz was working with another type of experiment, one that made it possible to isolate a single light pulse that lasted 650 attoseconds.
The laureates’ contributions have enabled the investigation of processes that are so rapid they were previously impossible to follow.
“We can now open the door to the world of electrons. Attosecond physics gives us the opportunity to understand mechanisms that are governed by electrons. The next step will be utilising them,” says Eva Olsson, Chair of the Nobel Committee for Physics.
There are potential applications in many different areas. In electronics, for example, it is important to understand and control how electrons behave in a material. Attosecond pulses can also be used to identify different molecules, such as in medical diagnostics.
Pierre Agostini. PhD 1968 from Aix-Marseille University, France. Professor at The Ohio State University, Columbus, USA.
Ferenc Krausz, born 1962 in Mór, Hungary. PhD 1991 from Vienna University of Technology, Austria. Director at Max Planck Institute of Quantum Optics, Garching and Professor at Ludwig-Maximilians-Universität München, Germany.
Anne L’Huillier, born 1958 in Paris, France. PhD 1986 from University Pierre and Marie Curie, Paris, France. Professor at Lund University, Sweden.
Understand: Electrons in pulses of light
A tiny hummingbird can beat its wings 80 times per second. We are only able to perceive this as a whirring sound and blurred movement. For the human senses, rapid movements blur together, and extremely short events are impossible to observe. We need to use technological tricks to capture or depict these very brief instants.
High-speed photography and strobe lighting make it possible to capture detailed images of fleeting phenomena. A highly focused photograph of a hummingbird in flight requires an exposure time that is much shorter than a single wingbeat. The faster the event, the faster the picture needs to be taken if it is to capture the instant.
The same principle applies to all the methods used to measure or depict rapid processes; any measurement must be done more quickly than the time it takes for the system being studied to undergo a noticeable change, otherwise, the result is vague. This year’s laureates have conducted experiments that demonstrate a method for producing pulses of light that are brief enough to capture images of processes inside atoms and molecules.
Atoms’ natural time scale is incredibly short. In a molecule, atoms can move and turn in millionths of a billionth of a second, femtoseconds. These movements can be studied with the very shortest pulses that can be produced with a laser – but when entire atoms move the timescale is determined by their large and heavy nuclei, which are extremely slow compared to light and nimble electrons.
When electrons move inside atoms or molecules, they do it so quickly that changes are blurred out in a femtosecond. In the world of electrons, positions and energies change at speeds of between one and a few hundred attoseconds, where an attosecond is one billionth of a billionth of a second.
An attosecond is so short that that the number of them in one second is the same as the number of seconds that have elapsed since the universe came into existence, 13.8 billion years ago. On a more relatable scale, we can imagine a flash of light being sent from one end of a room to the opposite wall – this takes ten billion attoseconds.
A femtosecond was long regarded as the limit for the fashes of light it was possible to produce. Improving existing technology was not enough to see processes occurring on the amazingly brief timescales of electrons; something entirely new was required. This year’s laureates conducted experiments that opened up the new research field of attosecond physics.
Shorter pulses with the help of high overtones Light consists of waves – vibrations in electrical and magnetic fields – that move through a vacuum faster than anything else. These have different wavelengths, equivalent to different colours. For example, red light has a wavelength of about 700 nanometres, one hundredth the width of a hair, and it cycles at about four hundred and thirty thousand billion times per second. We can think of
the shortest possible pulse of light as the length of a single period in the light wave, the cycle where it swings up to a peak, down to a trough, and back to its starting point. In this case, the wavelengths used in ordinary laser systems are never able to get below a femtosecond, so in the 1980s this was regarded as a hard limit for the shortest possible bursts of light.
The mathematics that describes waves demonstrates that any waveform can be built if enough waves of the right sizes, wavelengths and amplitudes (distances between peaks and troughs) are used. The trick to attosecond pulses is that it is possible to make shorter pulses by combining more and shorter wavelengths.
Observing electrons’ movements on an atomic scale requires short enough pulses of light, which means combining short waves of many different wavelengths. To add new wavelengths to light, more than just a laser is necessary; the key to accessing the briefest
instant ever studied is a phenomenon that arises when laser light passes through a gas. The light interacts with its atoms and causes overtones – waves that complete a number of entire cycles for each cycle in the original wave. We can compare this to the overtones that give a sound its particular character, allowing us to hear the difference between the same note played on a guitar and a piano.
In 1987, Anne L’Huillier and her colleagues at a French laboratory were able to produce and demonstrate overtones using an infrared laser beam that was transmitted through a noble gas. The infrared light caused more and stronger overtones than the laser with shorter wavelengths that had been used in previous experiments. In this experiment, many overtones of about the same light intensity were observed.
In a series of articles, L’Huillier continued to explore this effect during the 1990s, including at her new base, Lund University. Her results contributed to the theoretical understanding of this phenomenon, laying the foundation for the next experimental breakthrough.
Escaping electrons create overtones
When the laser light enters the gas and affects its atoms, it causes electromagnetic vibrations that distort the electric field holding the electrons around the atomic nucleus. The electrons can then escape from the atoms. However, the light’s electrical field vibrates continuously and, when it changes direction, a loose electron may rush back to its atom’s nucleus. During the electron’s excursion, it collected lots of extra energy from the laser light’s electrical field and, to reattach to the nucleus, it must release its excess energy as a pulse of light. These light pulses from the electrons are what create the overtones that appear in the experiments
Light’s energy is associated with its wavelength. The energy in the emitted overtones is equivalent to ultraviolet light, which has shorter wavelengths than the light visible to the human eye. Because the energy comes from the laser light’s vibrations, the overtones’ vibration will be elegantly proportional to the wavelength of the original laser pulse. The result of the light’s interaction with many different atoms is different light waves with a set of specific wavelengths.
Once these overtones exist, they interact with each other. The light becomes more intense when the lightwaves’ peaks coincide but becomes less intense when the peak in one cycle coincides with the trough of another. In the right circumstances, the overtones coincide so that a series of pulses of ultraviolet light occur, where each pulse is a few hundred attoseconds long. Physicists understood the theory behind this in the 1990s, but the breakthrough in actually identifying and testing the pulses occurred in 2001.
Pierre Agostini and his research group in France succeeded in producing and investigating a series of consecutive light pulses, like a train with carriages. They used a special trick, putting the “pulse train” together with a delayed part of the original laser pulse, to see how the overtones were in phase with each other. This procedure also gave them a measurement for the duration of the pulses in the train, and they could see that each pulse lasted just 250 attoseconds.
At the same time, Ferenc Krausz and his research group in Austria were working on a technique that could select a single pulse – like a carriage being uncoupled from a train and switched to another track. The pulse they succeeded in isolating lasted 650 attoseconds and the group used it to track and study a process in which electrons were pulled away from their atoms.
These experiments demonstrated that attosecond pulses could be observed and measured and that they could also be used in new experiments. Now that the attosecond world has become accessible, these short bursts of light can be used to study the movements of electrons. It is now possible to produce pulses down to just a few dozen attoseconds, and this technology is developing all the time.
Electrons’ movements have become accessible Attosecond pulses make it possible to measure the time it takes for an electron to be tugged away from an atom, and to examine how the time this takes depends on how tightly the electron is bound to the atom’s nucleus. It is possible to reconstruct how the distribution of electrons oscillates from
side to side or place to place in molecules and materials; previously their position could only be measured as an average.
Attosecond pulses can be used to test the internal processes of matter and to identify different events. These pulses have been used to explore the detailed physics of atoms and molecules, and they have potential applications in areas from electronics to medicine.
For example, attosecond pulses can be used to push molecules, which emit a measurable signal. The signal from the molecules has a special structure, a type of fingerprint that reveals what molecule it is, and the possible applications of this include medical diagnostics.
Facts about Nobel Prize in Physics
117 Nobel Prizes in Physics have been awarded between since 1901.
47 physics prizes have been given to one laureate only.
5 women have been awarded the physics prize so far: Marie Curie in 1903, Maria Goeppert-Mayer in 1963, Donna Strickland in 2018, Andrea Ghez in 2020 and Anne L’Huillier in 2023.
1 person, John Bardeen, has been awarded the physics prize twice. John Bardeen is the only person who has received the Nobel Prize in Physics twice, year 1956 and 1972 . Marie Curie was awarded the Nobel Prize twice, once in physics 1903 and once in chemistry 1911.
25 years was the age of the youngest physics laureate ever, Lawrence Bragg, when he was awarded the 1915 physics prize together with his father.
96 years was the age of the oldest physics laureate, Arthur Ashkin.
Married couples:
Marie Curie and Pierre Curie were awarded the Nobel Prize in Physics in 1903. Marie Curie was awarded the Nobel Prize a second time in 1911, this time receiving the Nobel Prize in Chemistry. (One of Marie and Pierre Curie’s daughters, Irène Joliot-Curie , was awarded the Nobel Prize in Chemistry in 1935 together with her husband Frédéric Joliot.)
Father and son: (All awarded the Nobel Prize in Physics.)
William Bragg and Lawrence Bragg, 1915
Niels Bohr, 1922 and Aage N. Bohr, 1975
Manne Siegbahn, 1924 and Kai M. Siegbahn, 1981
J. J. Thomson, 1906 and George Paget Thomson, 1937